Higgs Boson
Higgs Boson lucas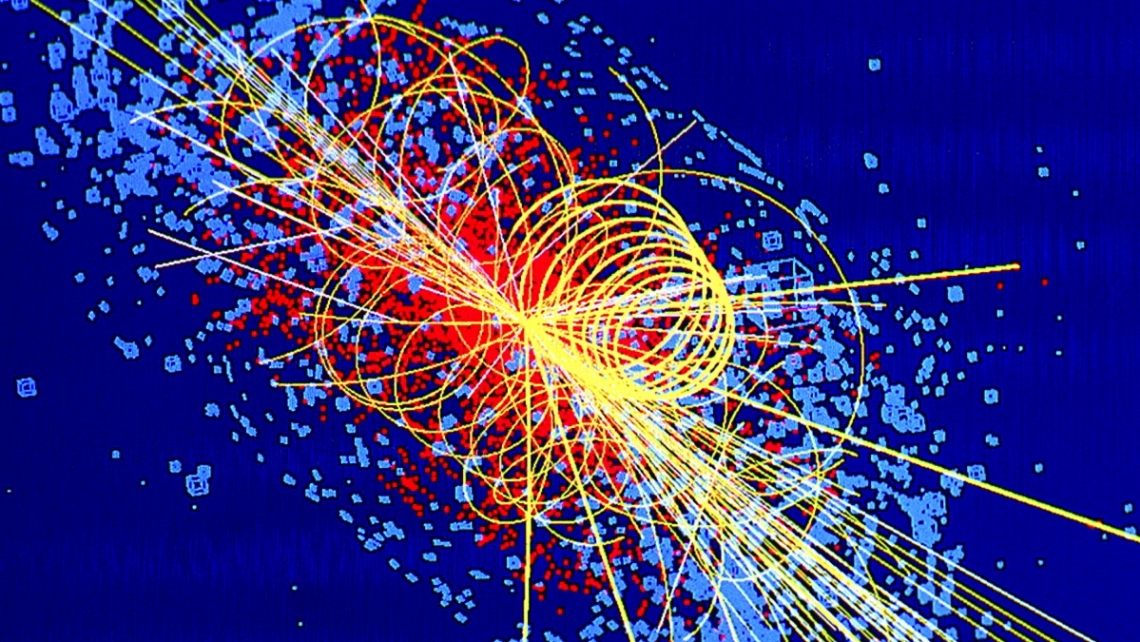
The Higgs boson is the fundamental particle predicted by the Brout-Englert-Higgs mechanism. This theory explains how fundamental particles acquire their mass. The search for the Higgs boson is one of the most fascinating scientific adventures. It started about 50 years ago and was considered impossible for decades, which is one of the main reasons the LHC was built.
The need for the Higgs
Our Universe seems to be described by four fundamental forces: gravity, electromagnetism, the weak force (which regulates nuclear phenomena like fusion within stars), and the strong force (which keeps atomic nuclei together). From around the turn of the last century, physicists have tried to unify these forces under a single, overarching theory. A major breakthrough occurred five decades ago when physicists realized that there are very close ties between the weak and electromagnetic forces. These two forces can be described by a single theory: electricity, magnetism, light, and some types of radioactivity are all manifestations of a single underlying force called the electroweak force.
The theory of electroweak interactions and Quantum Chromodynamics (the theory of the strong force) form the basis of the Standard Model. The Standard Model successfully describes all of the elementary particles we know and how they interact with one another. But our understanding of Nature is incomplete. In particular, the Standard Model as originally conceived could not explain one basic observation: why most elementary particles have mass. The symmetry responsible for electroweak unification requires the force-carrying particles involved to have no mass. The photon, carrier of the electromagnetic force, is found to follow that precept; however, the W and Z bosons, carriers of the weak force, have a mass. The fact that the W and Z boson are massive breaks the fundamental electroweak symmetry. It also leads, without corrections, to nonsensical predictions – for example, interactions with probabilities greater than 100%.
Needing a way out of this problem, several physicists[1] proposed a mechanism to account for the broken symmetry. Once incorporated into the equations, this electroweak-symmetry breaking mechanism would allow particles to have mass. The mechanism also explains why the weak interactions appear weak at low energies; the force carriers are massive, and therefore the force is correspondingly short-ranged. The Brout-Englert-Higgs mechanism requires the existence of a new particle, which we now call the Higgs boson.
According to our current understanding, all particles were massless just after the Big Bang. As the Universe cooled and the temperature fell below a critical value, an invisible field called the ‘Higgs field’ was formed; this field prevails throughout the cosmos. Particles such as the W and Z bosons acquire mass through their interaction with this field – the more intensely they interact, the heavier they become. The existence of the Brout-Englert-Higgs field explains the difference between the massless photon and the massive W and Z boson as we observe in Nature today. Other force-carrying particles – the photon and the gluon – do not have an interaction with the Higgs field and remain massless. The Higgs boson is the quantum particle associated with the Higgs field, just as the photon is the quantum particle associated with the electromagnetic field. Since the field cannot be observed directly, the LHC experiments search for its manifestation, the particle. The discovery of the particle proves the existence of the field.
Where does all mass come from?
Interactions with the Higgs field are not just reserved for force-carrying particles. The theory was extended to the rest masses of all other fundamental particles – such as the electron, or the quarks inside protons and neutrons. Composite particles, however, such as the protons and neutrons themselves, gain mass mainly through the binding energy holding them together. Without mass, the universe would be a very different place. For example, if the electron had no mass, there would be no atoms. Hence there would be no complex matter as we know it, no molecules, no chemistry, no biology, and no people. |
The particle hunt
The Higgs boson is the only fundamental particle predicted by the Standard Model that had not yet been seen by experiments when the LHC started. As for the other particles, the Standard Model does not predict the exact mass of the Higgs boson. Moreover, the probability of producing a Higgs boson is very low, making the particle more difficult to pin down. We had to look for it by systematically searching over a very large range of possible mass values. Fortunately, depending on its mass, the Standard Model Higgs boson was expected to leave characteristic footprints. The CMS experiment was built as a general purpose detector also for this purpose: to be sensitive to any possible Higgs mass. So we knew what to look for and starting from the measured particles in the detector, estimate its mass. If it turned out that we could not find it, this would have left the field wide open for physicists to develop a completely new theory to explain the origin of particle mass. The Higgs boson was at the top of physicists’ most-wanted list for more than four decades. However, in its most basic form, incorporating the Higgs field into the Standard Model is not completely satisfying. It does the job of explaining how the symmetry between electromagnetic and weak force-carriers is broken and it accounts for how force-carriers acquire their mass; but it does not predict or explain the degree of interaction with the field and hence the relative masses of these particles. Moreover, it does not explain why symmetry is broken in this way, nor does it predict the pattern of masses of quarks and leptons.
Other bosons – Looking beyond the Standard Model
We might find that the Higgs boson is different from the simplest version the Standard Model predicts. Many theories that describe physics beyond the Standard Model, such as Supersymmetry and compositeness models, suggest the existence of a zoo of new particles, including different kinds of Higgs bosons. CMS is a general-purpose detector. This means that not only is it designed with particular hypotheses in mind, but also with the aim to study whatever happens when particles collide at high energies, even if the results are like nothing we expect. If unexpected phenomena do occur, we are looking for them. It seems we are looking at just the visible tip of an iceberg – hidden below the Standard Model must be a deeper, more fundamental theory that gives reason to what we see on the surface.
References
[1] F. Englert and R. Brout, “Broken symmetry and the mass of gauge vector mesons”, Phys.Rev. Lett. 13 (1964) 321–323, doi:10.1103/PhysRevLett.13.321. P.W. Higgs, “Broken symmetries and the masses of gauge bosons”, Phys. Rev. Lett. 13 (1964) 508–509, doi:10.1103/PhysRevLett.13.508. G. Guralnik, C. Hagen, and T. W. B. Kibble, “Global conservation laws and massless particles”, Phys. Rev. Lett. 13 (1964) 585–587, doi:10.1103/PhysRevLett.13.585. It is also of note that Landau and Ginzburg had proposed a field giving the photon a mass in a superconductor, the maths of which is identical to the “Higgs mechanism” and predates it by several years. Credit: this text is based on a CERN "backgrounder" article and a CERN Bulletin article.
CMS Higgs Results
CMS Higgs Results lucasCMS Higgs Physics Results
Since the observation of the Higgs boson and establishing the couplings between the Higgs boson and photons, Z-bosons, and W-bosons, CMS physicists have made huge strides in the quest to better understand the Higgs boson.
With the data collected in 2016, we were able to observe the decay of the Higgs boson to tau leptons - strengthening previous results from combining CMS and ATLAS measurements that show the Higgs boson coupling to fermions (https://cds.cern.ch/record/2276465?ln=en )
Using the same data set, the production of the Higgs boson via top-antitop quark associated production was also confirmed, showing that the Higgs boson interacts with quarks as well as with leptons ( https://cms.cern/news/observation-tth-production )
In 2018, we observed the decay of the Higgs boson to pairs of b-quarks, completing the observation of the Higgs boson's interactions with all three of the heaviest fermions (https://cms.cern/news/higgs-observed-decaying-b-quarks-submitted )
Having confirmed the couplings between the Higgs boson and the three heaviest fermions, CMS also showed evidence for the interaction between the Higgs boson and the muon, the lighter cousin of the tau lepton. (https://cms.cern/news/cms-sees-evidence-higgs-boson-decaying-muons)
By combining measurements in the diphoton and four-lepton decay channels, we were also able to measure the mass of the Higgs boson with unprecedented precision of 0.1%! ( https://cms.cern/news/cms-precisely-measures-mass-higgs-boson - note that the article is based on a preliminary result; the published mass value is 125.38 ± 0.14 GeV)
Recently we have also measured the width of the Higgs boson by studying its decay into two Z-bosons: https://cms.cern/news/life-higgs-boson
ATLAS and CMS combine summer '11 search limits on the Standard Model Higgs
ATLAS and CMS combine summer '11 search limits on the Standard Model Higgs achintya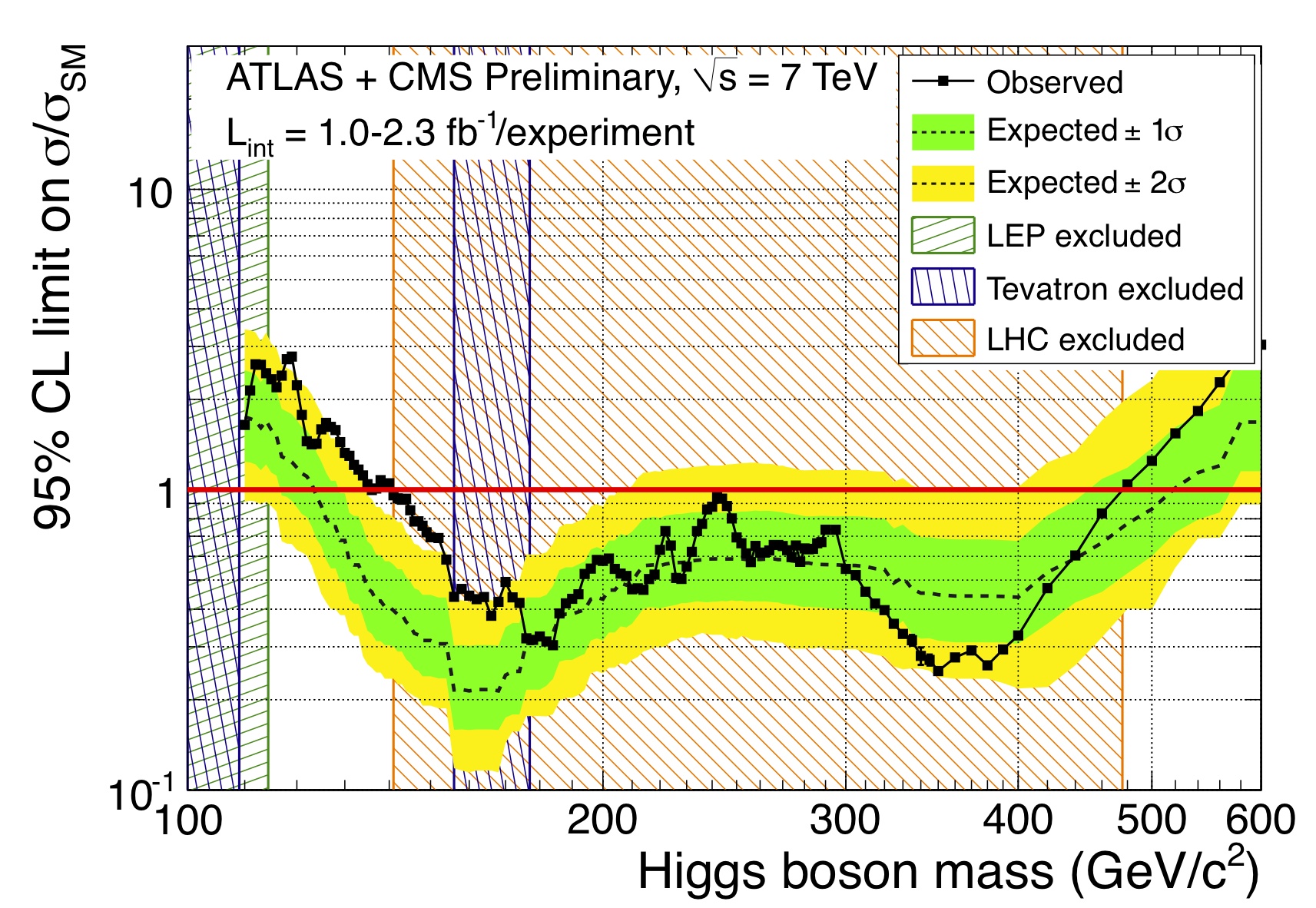
Perhaps the most anticipated result of the LHC involves the search for the Higgs boson, the only particle predicted by the Standard Model (SM) that has not yet been seen by experiments. The Higgs boson helps explain how elementary particles acquire mass. If the SM Higgs boson exists it will be produced at the LHC and swiftly decay into various known and well-studied particles, with the dominant decay products depending on the actual Higgs mass. ATLAS and CMS search for the SM Higgs boson using a range of decay products: two photons; two tau leptons; two b quarks; two W bosons; and two Z bosons. Analysing all these channels ensures that the search is sensitive to observing the Higgs irrespective of its mass. First results from ATLAS and CMS on searches for the SM Higgs boson with about 1 inverse femtobarn[1] (fb−1) of data were reported already in July at the EPS2011 meeting in Grenoble, and updates were shown one month later at the Lepton Photon 2011 conference in Mumbai with data samples of up to 2.3 fb−1. By combining the individual results of the two experiments an increased sensitivity in the search can be obtained. Hence, as a next logical step, the experiments have now combined the search data released this summer. A team of physicists from ATLAS and CMS was put in place early this year to prepare for such a combination. Correlations between the individual results have been carefully taken into account. Various statistical combination techniques have been explored to perform the combination, and a huge number of cross checks were made in the last few months. Finally, the combined search result is now ready. The results of the combination are shown in the figure, which depicts in a very clear way the enormous impact of the 2011 LHC data on the search for the SM Higgs boson. The CMS and ATLAS combined search excludes at >95% confidence level[2] the presence of the Standard Model Higgs in the mass range[3] 141-476 GeV. Indeed, the region from 146 to 443 GeV is excluded at 99% confidence level with the exception of three small regions between 220 and 320 GeV. Nonetheless, exploration continues of the region at low mass that is most favoured by indirect measurements, as the experiments collect and analyse more data to increase their sensitivity. Clearly this region will be the main focus of the searches with the complete 2011 data sample, which amounts to more than 5 fb−1 recorded by each of the experiments, and in 2012. At the same time, the search in the full range will continue to look for production of non-Standard Model Higgs bosons, with a possible lower production rate in the channels studied so far, and by exploring new decay channels. The next few months are definitely going to be very exciting. More information can be found in CMS-PAS-HIG-11-023, ATLAS-CONF-2011-157.
Video
Follow the combination process from the CMS perspective (includes exclusive footage from internal CMS meetings):
Additional information
- All CMS Higgs plots and results
- All CMS Papers
- All CMS Physics Analysis Summaries
- All CMS results
- Images of real collisions in CMS
- Animations of real collisions in CMS
About CMS
CMS is one of two general-purpose experiments at the LHC that have been built to search for new physics. It is designed to detect a wide range of particles and phenomena produced in the LHC's high-energy proton-proton and heavy-ion collisions and will help to answer questions such as: "What is the Universe really made of and what forces act within it?" and "What gives everything substance?" It will also measure the properties of well-known particles with unprecedented precision and be on the lookout for completely new, unpredicted phenomena. Such research not only increases our understanding of the way the Universe works, but may eventually spark new technologies that change the world in which we live as has often been true in the past. The conceptual design of the CMS experiment dates back to 1992. The construction of the gigantic detector (15 m diameter by nearly 29 m long with a weight of 14000 tonnes) took 16 years of effort from one of the largest international scientific collaborations ever assembled: more than 3100 scientists and engineers from 169 institutions and research laboratories distributed in 39 countries all over the world. For further information, contact: cms.outreach@cern.ch.
- [1] http://news.stanford.edu/news/2004/july21/femtobarn-721.html
http://www.quantumdiaries.org/2011/03/02/why-don%E2%80%99t-we-just-say-collision-rate/ - [2] Confidence level is a statistical measure of the number of times out of 100 that test results can be expected to be within a specified range. For example, a confidence level of 95% means that the result of an action will probably meet expectations 95% of the time. (Source: NADbank)
- [3] By mass-energy equivalence, the electron volt is also a unit of mass. It is common in particle physics, where mass and energy are often interchanged, to use eV/c2, where c is the speed of light in a vacuum (from E = mc2). Even more common is to use a system of natural units with c set to 1 (hence, E = m), and simply use eV as a unit of mass. (Source: Wikipedia)
CMS search for the Standard Model Higgs Boson in LHC data from 2010 and 2011
CMS search for the Standard Model Higgs Boson in LHC data from 2010 and 2011 lucas![Figure 1: A typical candidate event including two high-energy photons whose energy (depicted by dashed yellow lines and red towers) is measured in the CMS electromagnetic calorimeter. The yellow lines are the measured tracks of other particles produced in the collision. <a href="https://cdsweb.cern.ch/record/1406073">[ See more event display images. ]</a>](/sites/default/files/field/image/gg-run177878-evt188723900-3d.jpg)
CERN, 13th December 2011
The statement below is also available as PDF files in: Chinese (traditional) | Chinese (simplified) | Croatian | Dutch | English | Finnish | French | German | Greek | Hungarian | Italian | Persian | Polish | Spanish | Portuguese | Russian | Serbian | Turkish
The Higgs boson is the only particle predicted by the Standard Model (SM) of particle physics that has not yet been experimentally observed. Its observation would be a major step forward in our understanding of how particles acquire mass. Conversely, not finding the SM Higgs boson at the LHC would be very significant and would lead to a greater focus on alternative theories that extend beyond the Standard Model, with associated Higgs-like particles. Today the CMS Collaboration presented their latest results in the search for the Standard Model Higgs boson, using the entire data sample of proton-proton collisions collected up to the end of 2011. These data amount to 4.7 fb-1 of integrated luminosity[1], meaning that CMS can study Higgs production in almost the entire mass range above the limit from CERN’s Large Electron Positron (LEP) collider of 114 GeV/c2 (or 114 GeV in natural units [2]) and up to 600 GeV. Our results were achieved by combining searches in a number of predicted Higgs “decays channels” including: pairs of W or Z bosons, which decay to four leptons; pairs of heavy quarks; pairs of tau leptons; and pairs of photons (Figure 1). Our preliminary results, for several statistical confidence levels [3], exclude the existence of the SM Higgs boson in a wide range of possible Higgs boson masses:
- 127 – 600 GeV at 95% confidence level, as shown in Figure 2a; and
- 128 – 525 GeV at 99% confidence level.
A mass is said to be “excluded at 95% confidence level” if the Standard Model Higgs boson with that mass would yield more evidence than that observed in our data at least 95% of the time in a set of repeated experiments. We do not exclude a SM Higgs boson with a mass between 115 GeV and 127 GeV at 95% confidence level. Compared to the SM prediction there is an excess of events in this mass region (see Figure 2b), that appears, quite consistently, in five independent channels. With the amount of data collected so far, it is inherently difficult to distinguish between the two hypotheses of existence vs non-existence of a Higgs signal in this low mass region. The observed excess of events could be a statistical fluctuation of the known background processes, either with or without the existence of the SM Higgs boson in this mass range. The larger data samples to be collected in 2012 will reduce the statistical uncertainties, enabling us to make a clear statement on the possible existence, or not, of the SM Higgs boson in this mass region. The excess is most compatible with an SM Higgs hypothesis in the vicinity of 124 GeV and below, but with a statistical significance of less than 2 standard deviations (2σ) from the known backgrounds, once the so-called Look-Elsewhere Effect [4] has been taken into account. This is well below the significance level that traditionally has been associated with excesses that stand the test of time. If we explore the hypothesis that our observed excess could be the first hint of the presence of the SM Higgs boson, we find that the production rate (“cross section” relative to the SM, σ/σSM) for each decay channel is consistent with expectations, albeit with large uncertainties. However, the low statistical significance means that this excess can reasonably be interpreted as fluctuations of the background. More data, to be collected in 2012, will help ascertain the origin of the excess. UPDATE: CMS publishes Higgs boson search results using 2010–2011 data
- This statement is available online at:
http://cern.ch/cms/news/cms-search-standard-model-higgs-boson-lhc-data-2010-and-2011 - For more details see the CMS public website: http://cern.ch/cms
Event images and animations of real CMS data
- Collision event display images from the Higgs analysis of 2010 and 2011 data
- Collision event animation (4 electrons) from the Higgs analysis of 2010 and 2011 data
- Collision event animation (2 photon) from the Higgs analysis of 2010 and 2011 data
- Collision event animation (4 muons) from the Higgs analysis of 2010 and 2011 data
Short movies about the Higgs
- Animation of the Higgs mechanism [ with subtitles] See also [ without subtitles]
- What is the Higgs? by Don Lincoln
- Higgs Boson: How do we search for it? by Don Lincoln
Seminar at CERN, 13th December 2011
- CMS seminar slides (Prof. G. Tonelli)
- CERN Press Release, 13th December 2011 [ Also available in French ]
- ATLAS Experiment Higgs results
More about the Higgs at CMS
- CMS Physics Analysis summary papers about the Higgs
- Simple explanations of Higgs search terminology
- Search for the Standard Model Higgs Boson in CMS
- All CMS Higgs plots and results
About CMS Physics
- All CMS Papers
- All CMS Physics Analysis Summaries
- All CMS results
- Images of real collisions in CMS
- Animations of real collisions in CMS
About CMS
More information may be found on the CMS web site: http://cern.ch/cms. CMS is one of two general-purpose experiments at the LHC that have been built to search for new physics. It is designed to detect a wide range of particles and phenomena produced in the LHC's high-energy proton-proton and heavy-ion collisions and will help to answer questions such as: "What is the Universe really made of and what forces act within it?" and "What gives everything substance?" It will also measure the properties of well-known particles with unprecedented precision and be on the lookout for completely new, unpredicted phenomena. Such research not only increases our understanding of the way the Universe works, but may eventually spark new technologies that change the world in which we live as has often been true in the past. The conceptual design of the CMS experiment dates back to 1992. The construction of the gigantic detector (15 m diameter by nearly 29 m long with a weight of 14000 tonnes) took 16 years of effort from one of the largest international scientific collaborations ever assembled: more than 3100 scientists and engineers from 169 institutions and research laboratories distributed in 39 countries all over the world. For further information, contact: cms.outreach@cern.ch.
Footnotes
- [1] http://news.stanford.edu/news/2004/july21/femtobarn-721.html
- [2] The electron volt is a unit of energy. In particle physics, where mass and energy are often interchanged, it is common to use eV/c2 as a unit of mass (from E = mc2, where c is the speed of light in vacuum). Even more common is to use a system of natural units with c set to 1 (hence, E = m), and simply use eV as a unit of mass.
- [3] Confidence level is a statistical measure of the percentage of test results that can be expected to be within a specified range. For example, a confidence level of 95% means that the result of an action will probably meet expectations 95% of the time.
- [4] http://cmsexperiment.web.cern.ch/news/should-you-get-excited-your-data-…
New CMS Higgs Search Results for the Lepton Photon 2011 Conference
New CMS Higgs Search Results for the Lepton Photon 2011 Conference lucas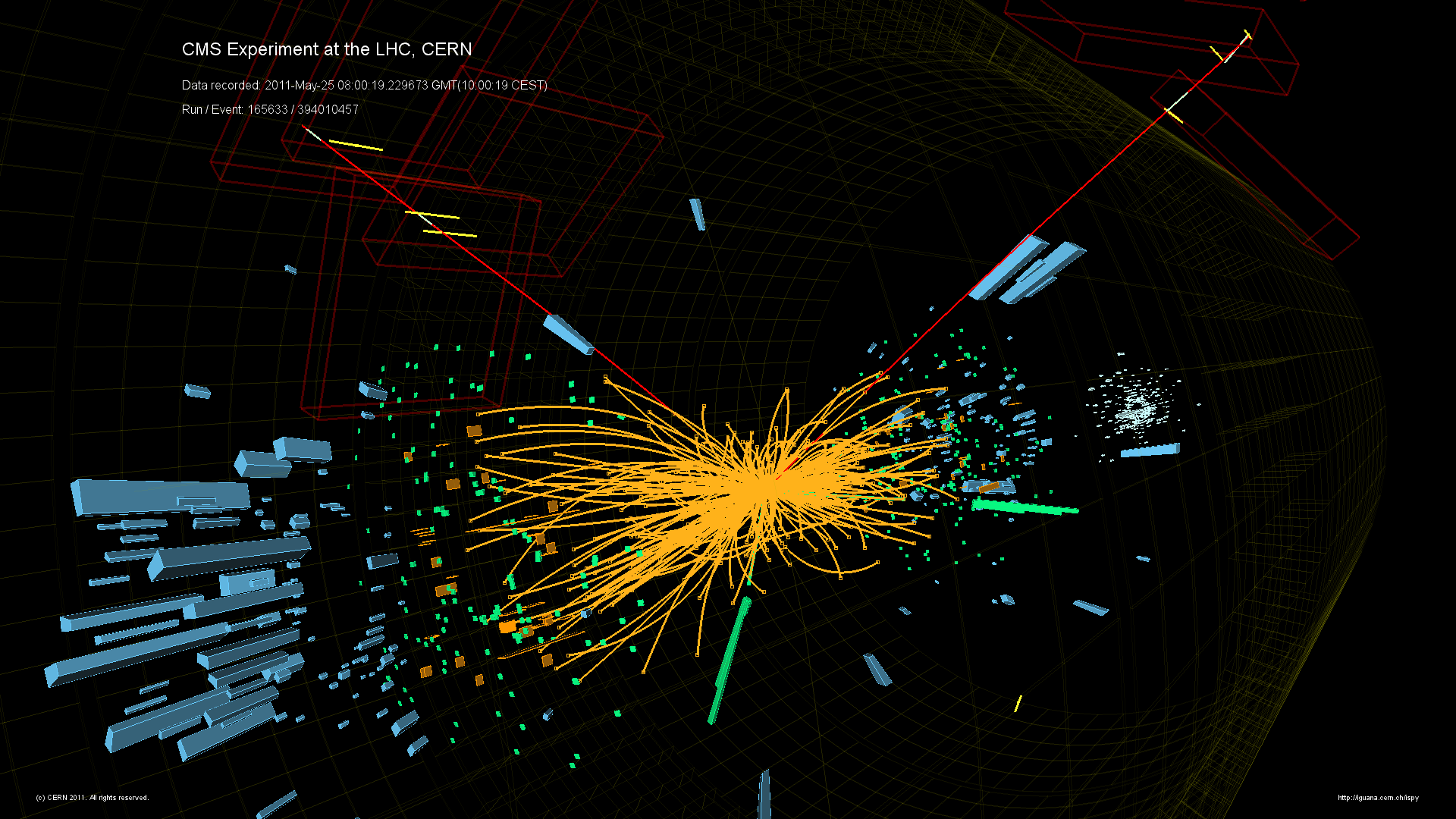
The Higgs boson is the only particle predicted by the Standard Model that has not yet been seen by experiments. It helps explain how elementary particles acquire mass. If the Higgs boson exists it will be produced in proton-proton collisions at the LHC. It then swiftly decays into various known and well-studied particles (depending on the actual Higgs mass). The CMS search for the Higgs boson is being carried out using a range of decay products: two photons; two tau leptons; two b quarks; two W bosons; and two Z bosons. Analysing all these channels ensures that the search is sensitive to observing the Higgs irrespective of its own mass. An example of an event passing the selection criteria is shown in Figure 1, in which two Z bosons are identified through their decays to a pair of electrons and a pair of muons respectively. These results are based on data recorded during LHC running at an energy of 7 TeV in 2010 and 2011. The analysis uses 1.1 – 1.7 inverse femtobarns[1] (integrated luminosity) of data, depending on the channel — an inverse femtobarn corresponds to about 100 trillion proton-proton collisions. CMS observes no convincing excess of events in the explored mass[2] range of 110-600 GeV (for full details see [HIG-11-022]). The analysis excludes, with a confidence level (C.L.) of 95%[3], the existence of a Standard Model Higgs boson in three Higgs mass ranges: 145-216 GeV, 226-288 GeV and 310-400 GeV (Figure 2). For the quantity of data we have collected, on average we would expect to exclude the range 130-440 GeV in the absence of a signal. We believe that the differences between the expected and observed exclusion mass ranges are consistent with statistical fluctuations. At 90% C.L., we exclude the SM Higgs boson in the mass range from 144-440 GeV. All exclusion regions were obtained using the modified frequentist construction confidence levels. It should be noted that a modest excess of events is observed for Higgs boson masses below 145 GeV. With the data we will collect in the coming months we will be able to distinguish between the possible interpretations: the production of a SM Higgs boson or a statistical fluctuation of the backgrounds. During the ongoing LHC proton-proton data-taking period, expected to terminate at the end of 2012, CMS will record substantially more data that should be sensitive to observing a SM Higgs boson, if it exists, over the full range of possible masses.
More information
- All CMS Papers (see also: timeline graphical index of all papers)
- All CMS Physics Analysis Summaries
- All CMS results
About CMS
More information, including images and animations of CMS collision events, may be found on the CMS web site: http://cms.cern.ch. CMS is one of two general-purpose experiments at the LHC that have been built to search for new physics. It is designed to detect a wide range of particles and phenomena produced in the LHC's high-energy proton-proton and heavy-ion collisions and will help to answer questions such as: "What is the Universe really made of and what forces act within it?" and "What gives everything substance?" It will also measure the properties of well-known particles with unprecedented precision and be on the lookout for completely new, unpredicted phenomena. Such research not only increases our understanding of the way the Universe works, but may eventually spark new technologies that change the world in which we live as has often been true in the past. The conceptual design of the CMS experiment dates back to 1992. The construction of the gigantic detector (15 m diameter by nearly 29 m long with a weight of 14000 tonnes) took 16 years of effort from one of the largest international scientific collaborations ever assembled: more than 3100 scientists and engineers from 169 institutions and research laboratories distributed in 39 countries all over the world. For further information, contact: cms.outreach@cern.ch.
- [1] http://news.stanford.edu/news/2004/july21/femtobarn-721.html
http://www.quantumdiaries.org/2011/03/02/why-don%E2%80%99t-we-just-say-collision-rate/ - [2] By mass-energy equivalence, the electron volt is also a unit of mass. It is common in particle physics, where mass and energy are often interchanged, to use eV/c2, where c is the speed of light in a vacuum (from E = mc2). Even more common is to use a system of natural units with c set to 1 (hence, E = m), and simply use eV as a unit of mass. (Source: Wikipedia)
- [3] Confidence level is a statistical measure of the number of times out of 100 that test results can be expected to be within a specified range. For example, a confidence level of 95% means that the result of an action will probably meet expectations 95% of the time. (Source: NADbank)
New CMS Results for the EPS 2011 Conference
New CMS Results for the EPS 2011 Conference lucas![Fig 1. Event display of a ZZ event. One Z decays to two electrons (red towers) [...]](/sites/default/files/field/image/Fig1-CMS-2e-2mu-evt-394010457-3D.jpg)
The CMS collaboration is presenting its latest results this week at the 2011 Europhysics Conference on High-Energy Physics, held in Grenoble, France. These results are based on about 1 inverse femtobarn[1] of data (100 trillion proton-proton collisions) from LHC running at an energy of 7 TeV, which were collected in 2010 and 2011. They include a wide range of searches for new physics and precise measurements of Standard Model processes.
Higgs Search
The Higgs boson is the only particle predicted by the Standard Model that has not yet been seen by experiments. It helps explain how elementary particles acquire mass. If the Higgs boson exists it will be produced in proton-proton collisions at the LHC and then swiftly decay into several known and well-studied particles. The CMS search for the Higgs boson is being carried out using a range of decay products: two photons; two tau leptons; two W bosons; and two Z bosons. The W is observed through its decay to an electron plus a neutrino, or a muon plus a neutrino. The Z is observed through its decay to a pair of electrons, or a pair of muons, (see Figure 1) or a pair of jets of hadronic particles. Analysing all these channels ensures that the search is sensitive to observing the Higgs irrespective of its own mass. CMS observes no convincing excesses of events in the explored mass[2] range of 120-600 GeV (for full details see [HIG-11-011]). The analysis excludes, with a confidence level of 95%[3], the existence of a Higgs boson in two broad Higgs mass ranges, 149-206 GeV and 300-440 GeV, as well as several narrower intervals in between (Figure 2). At a lower confidence level of 90%, the existence of a Higgs boson is excluded for the range 145-480 GeV. Re-interpreting the results in the context of the Standard Model with a fourth generation of fermions in addition to the known three generations (SM4), allows us to exclude the SM4 Higgs boson with a mass in the range 120-600 GeV with a confidence level of 95%. It should be noted that a modest excess of events is observed for Higgs boson masses below 145 GeV. With the data we will collect in the next few months we will be able to distinguish between the possible interpretations: the production of a Higgs boson or a statistical fluctuation of the backgrounds. During the ongoing LHC proton-proton run CMS will record substantially more data that should be sensitive to observing a Higgs boson, if it exists, over the full range of possible masses.
Search for rare decays of Bs→μμ
Bs mesons, comprising a 'bottom' and a 'strange' quark, are produced copiously at the LHC. The fraction that subsequently decays (known as the "branching fraction") to a pair of easily-detected muons is highly suppressed in the Standard Model — only about three such decays are expected per billion Bs particles produced. Several extensions of the SM, for instance Supersymmetric models, predict significant enhancements in the number of decays to pairs of muons, thanks to new particles that would contribute to the decay through "virtual loops". Therefore, any enhancement of this Bs decay rate could indicate the existence of new physics. CMS has searched for the decays of Bs (and B0 particles, comprising a 'bottom' and a 'down' quark) to muon pairs using proton-proton collision data collected up to June 2011 (Figure 3). A challenging aspect of this search is reducing the large backgrounds from other B-hadron decays or particles misidentified as muons. The number of candidate decays observed in the available data sample is consistent with the Standard Model expectations for signal and background (see Figure 4). Given the absence of a significant excess, CMS has excluded (at 95% confidence level) branching fractions larger than 1.9x10-8 and 4.6x10-9 for the decay of Bs and B0 particles, respectively. This result constitutes one of the most stringent exclusion limits achieved until now. The data CMS will collect in the remainder of 2011 and in 2012 will be sensitive to smaller branching fractions, at the level of SM expectations, and may lead to the observation of an enhanced decay rate which could be indicative of a non-Standard-Model physics process.
Other Results
CMS has searched for many other possible signatures of exotic new physics. No significant signals for new physics have yet been seen and therefore new constraints, at 95% confidence level, have been placed on many extensions to the Standard Model, including the following:
- The Supersymmetry (SUSY) theory provides a symmetry between matter and forces, and predicts that for each known particle there is a 'supersymmetric' partner. SUSY quarks, or squarks, are ruled out by CMS up to 1.2 TeV for a large range of SUSY parameters in the models tested [SUS-11-003].
- High-mass W′ and Z′ particles with Standard Model couplings are ruled out up to masses of 2.27 TeV [EXO-11-024] and 1.94 TeV [EXO-11-019] respectively. Constraints are placed on other models producing high-mass di-lepton resonances. Analysis of top-anti-top final states rules out the "Kaluza Klein gluon" (in Randal Sundrum models) with mass below 1.5 TeV [EXO-11-006].
- A study of di-jet resonances excludes excited quarks in the mass range of 1.0 TeV to 2.5 TeV and similarly constrains models involving E6 di-quarks, string resonances, the W′, axigluons and colorons [EXO-11-015].
- A search for the pair production of a fourth-generation t′ quark and its antiparticle ruled them out up to a t′ mass of 450GeV [EXO-11-051].
- Microscopic black-hole production is ruled out for black-hole masses up to between 4 and 5 TeV, depending on the model in question [EXO-11-071].
CMS also has a rich programme that confronts the predictions of the Standard Model with precise experimental measurements at the high-energy frontier of the LHC. These include top-quark production that has been studied in detail, with recent measurements made of the cross-section for top quark-top antiquark pairs decaying fully hadronically [TOP-11-007], the cross-section measurement using events with a tau lepton in the final state [TOP-11-006] and the top charge asymmetry [TOP-11-014]. Many aspects of the Electroweak and Strong forces as well as b-quarks are being studied. These analyses have already resulted in more than thirty publications in refereed journals, with many more results in the pipeline. A good understanding of Standard Model processes is also important as they contribute to the backgrounds to new physics processes.
Outlook
The LHC machine is running extremely well and has already delivered more proton-collision data than was expected in the entire 2011 run. The high-precision CMS experiment is recording good quality data with high efficiency. By the end of 2012 we expect to increase the size of our data sample by a factor of about ten. We are just starting to explore a vast territory where new physics could manifest itself and the CMS experiment is ready for whatever Nature has in store for us.
More information
- All CMS Papers (see also: timeline graphical index of all papers)
- All CMS Physics Analysis Summaries
- All CMS results
About CMS
More information, including images and animations of CMS collision events, may be found on the CMS web site: http://cms.cern.ch. CMS is one of two general-purpose experiments at the LHC that have been built to search for new physics. It is designed to detect a wide range of particles and phenomena produced in the LHC's high-energy proton-proton and heavy-ion collisions and will help to answer questions such as: "What is the Universe really made of and what forces act within it?" and "What gives everything substance?" It will also measure the properties of well-known particles with unprecedented precision and be on the lookout for completely new, unpredicted phenomena. Such research not only increases our understanding of the way the Universe works, but may eventually spark new technologies that change the world in which we live as has often been true in the past. The conceptual design of the CMS experiment dates back to 1992. The construction of the gigantic detector (15 m diameter by nearly 29 m long with a weight of 14000 tonnes) took 16 years of effort from one of the largest international scientific collaborations ever assembled: more than 3100 scientists and engineers from 169 institutions and research laboratories distributed in 39 countries all over the world. For further information, contact: cms.outreach@cern.ch.
- [1] http://news.stanford.edu/news/2004/july21/femtobarn-721.html
http://www.quantumdiaries.org/2011/03/02/why-don%E2%80%99t-we-just-say-collision-rate/ - [2] By mass-energy equivalence, the electron volt is also a unit of mass. It is common in particle physics, where mass and energy are often interchanged, to use eV/c2, where c is the speed of light in a vacuum (from E = mc2). Even more common is to use a system of natural units with c set to 1 (hence, E = m), and simply use eV as a unit of mass. (Source: Wikipedia)
- [3] Confidence level is a statistical measure of the number of times out of 100 that test results can be expected to be within a specified range. For example, a confidence level of 95% means that the result of an action will probably meet expectations 95% of the time. (Source: NADbank)
Higgs Boson -- Terms and Definitions
Higgs Boson -- Terms and DefinitionsBackground
When scientists search for new physics, they compare what they observe to what theories predict they will observe. The background is the set of results scientists expect to see. If an experiment sees more instances of a certain type of event (see “Excess”) than they expect to see as part of the background, it might be evidence of new physics.
Confidence Level (CL)
Confidence level is a statistical measure of the percentage of test results that can be expected to be within a specified range. For example, a confidence level of 95 percent means that the result of an action will probably meet expectations 95 percent of the time. When physicists search for a signal of the Higgs boson, they select particle collisions with observed characteristics similar to those a Higgs production would feature. The result of this data selection will always include background events, i.e. ones which did not involve a Higgs boson decay, but are anyway individually undistinguishable from the signal. The existence of the Higgs boson would however increase the number of selected events, so one may compare the number of selected events with the predicted yield from known backgrounds alone, to make a statistical statement about the Higgs production rate. If the number of observed events is close to the background prediction, then one can rule out a sizable contribution from the Higgs boson decay signal, which would have created an excess of events. Given a signal rate and a background prediction, one can compute the probability that a total number of events smaller or equal to the ones observed is seen. Signal rates for which the probability would be smaller than 5% are then said to be "excluded at 95% confidence level". Note that the choice of 95% as a division line between what is allowed or excluded by the data is arbitrary, and has merely historical justification.
Decay channel
Most massive particles like the Higgs boson are unstable and decay into other particles over time. Just as a vending machine might return the same amount of change using different combinations of coins, a particle can decay into different combinations of particles. These sets of secondary particles are called decay channels. If the Standard Model Higgs boson exists, it could decay into several different channels, such as two photons or two W bosons or two Z bosons. Physicists have calculated how often a Standard Model Higgs boson would decay into these different channels depending on its mass. They search for excesses of events in those decay channels, which could indicate the presence of a Higgs.
Event
An event is a snapshot of a collision in the LHC. Because energy is equivalent to mass, highly energetic collisions can create particles more massive than those involved in the collisions (protons, in the case of the LHC). These massive particles quickly decay into lighter stable particles (see “Decay channel”). Physicists study the decay products of collisions to determine what more massive particles were created in the events.
Excess
When scientists observe more of a certain type of event than expected in a data plot, they call that an excess. Scientists measure the statistical significance (See “Sigma” and “Standard deviation”) of excesses to determine how certain they are that they result from new physics and not simply random fluctuations.
Exclusion
If searches for a particle reveal statistically that it is unlikely to exist with certain characteristics (e.g. a particular mass), those characteristics can be excluded. This narrows the search parameters within which the particle might be found. Establishing such exclusions is important in the search for undiscovered particles.
Look-elsewhere effect (LEE)
When physicists see more of a certain type of event than predicted, they must consider the look-elsewhere effect in determining the statistical significance of that excess of events. To calculate the look-elsewhere effect for a certain observation, scientists take into account the probability of seeing something similar in any particular spot over the mass range in question. The chances of a statistical fluctuation causing an excess of events at one point on a plot are lower than the chances of a statistical fluctuation leading to an excess of at any point in a range of points on a plot. Other things being equal, the smaller the range one considers, the smaller the look-elsewhere effect. [Read more.]
Standard Deviation / sigma
The standard deviation describes the spread of a set of measurements around the mean value. It can be used to quantify the level of disagreement of a set of data from a given hypothesis. Physicists express standard deviations in units called “sigma”. The higher the number of sigma, the more incompatible the data are with the hypothesis. Typically, the more unexpected a discovery is, the greater the number of sigma physicists will require to be convinced.
Standard Model
The Standard Model is a collection of theories that embodies all of our current understanding about the behaviour of fundamental particles.
Blind analysis
To avoid bias when analysing new data, physicists perform the analysis “blind” — that is, without seeing how the application of various selection criteria would affect the result before the analysis is complete. [Read more.]
How is CMS searching for the Higgs Boson?
How is CMS searching for the Higgs Boson? lucas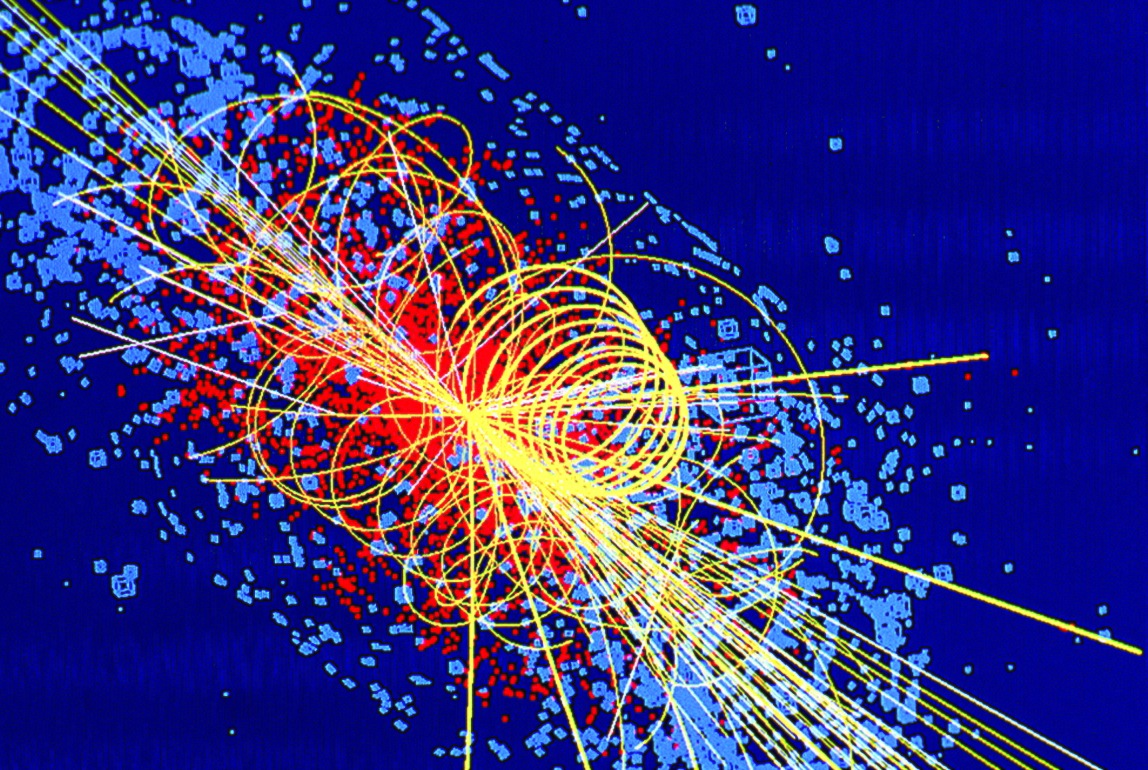
The discovery of the Higgs boson, one of the main goals of the CMS experiment, proved that the Higgs field exists. When a Higgs boson is formed in an LHC collision, it decays very quickly to other particles. Therefore, we have to study these decay products, the 'signature' the Higgs boson leaves behind, to learn about the Higgs boson. When the Higgs boson was first observed, it was seen in decays to pairs of Z-bosons, with subsequent decay into four leptons (particles such as electrons or muons), as well as in decays to pairs of photons.
The event shows characteristics expected from the decay of the SM Higgs boson to a pair of Z bosons, one of which subsequently decays to a pair of electrons (green lines and green towers) and the other Z decays to a pair of muons (red lines).
Photons are detected in the electromagnetic calorimeter. In the first image we see the detector head-on with the electromagnetic calorimeter (ECAL) in green. Green trajectories show these photons, while the blue trajectories show other particles emerging from the collision with the red marks showing hits in the hadron calorimeter. The ECAL is able to tell the mass of the particle to better than 1%.
Event recorded with the CMS detector in 2012 at a proton-proton centre of mass energy of 8 TeV. The event shows characteristics expected from the decay of the SM Higgs boson to a pair of photons (dashed yellow lines and green towers).
You can watch this video series to learn more about how the Higgs boson was discovered. https://www.youtube-nocookie.com/watch?v=so2nCu2Jkbc
Now that the Higgs boson has been discovered, at CMS, we're making further measurements of its properties. We're not just looking at the two discovery channels for this, but also at other major Higgs boson decay modes: into pairs of b-quarks, tau leptons, and W-bosons... If you'd like to know more, this video series sheds some more light on these different channels.
Blinding and unblinding analyses
Blinding and unblinding analyses achintya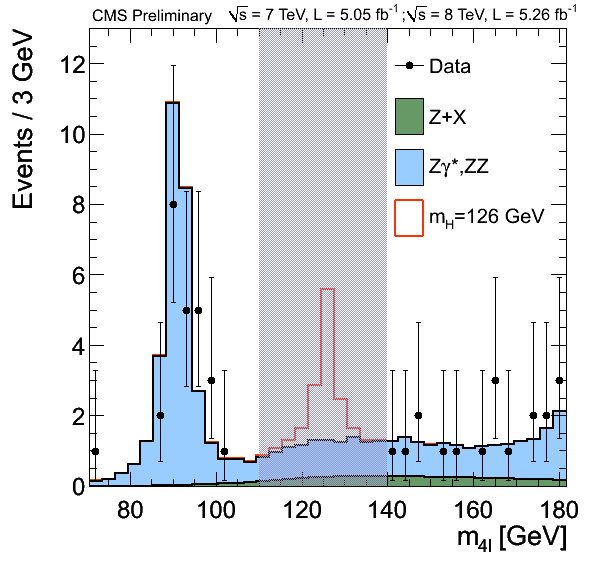
CMS performs searches for new particles by looking for signals amidst a background of known physics. If the data begin to indicate something more interesting than merely background - for instance, more decay events than expected in a certain region - it is important to make sure that the observation is statistically significant by collecting and analysing more data. However, we don't want to bias our analyses by optimising the analysis based on what was already seen. To avoid such bias while analysing new data, physicists draw "blinds” over the region where an excess of decay events is expected; this region is only "unblinded" when they are satisfied with their procedures. This ensures objectivity when it comes to looking for much-sought-after signs of new physics, and gives confidence in the ultimate result.
The procedure is similar to that used by medical researchers when testing a new treatment. Even though the Higgs boson was discovered a decade ago, we still employ this procedure for measuring the Higgs boson's properties - we still do not want to bias these measurements by optimising towards deviations from the standard model expectations! All of the available data, including those in the blinded region, are used in the analysis; you just don’t peek at this region to see how it changes as you tweak various selection criteria. In many of the on-going searches and measurements, data corresponding to a possible signal look very similar to data corresponding to background.
Therefore, once the analysis is blinded, physicists must optimise two things: 1) simulations, to help determine the criteria for selecting signals and 2) the methods for rejecting or quantifying background events using data from the region where there is no excess. To cross-check all the details, the various steps involved in the analysis procedures are usually carried out by at least two independent teams. If both teams see similar results in the background region, the analysis is signed off by the wider collaboration. It is only after the sign-off that the signal region is unblinded. The results of the unblinding are put through further scrutiny by the collaboration before being made public.
Should you get excited by your data? Let the Look-Elsewhere Effect decide
Should you get excited by your data? Let the Look-Elsewhere Effect decide dorigo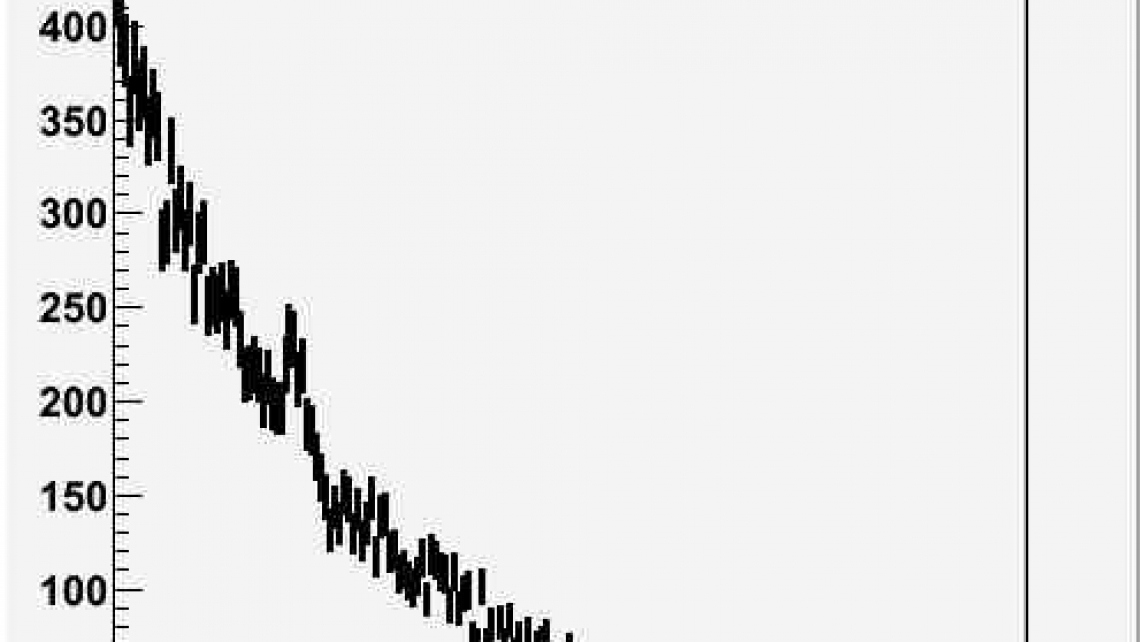
A dangerous beast is hiding in today’s searches for the rare signs of new physics — or even “old” physics, such as the Higgs boson — at the Large Hadron Collider. It is called “Look-Elsewhere Effect”, LEE for insiders. What is it, and why should you care? Imagine you look for a heavy particle decaying into a pair of hadronic jets: a commonplace test case in high-energy physics. You have your background model, which predicts the observable shape of the di-jet mass distribution, and you know what kind of a bump a new particle signal would produce in that shape. So you search for such a bump in the data, but — not knowing where it might appear — you search everywhere. You have worked all day, and the night is nearing; you prepare yourself a martini and spin your analysis program. To your amazement, the program finds a significant bump at some particular mass value: is it a real signal? To claim it is a new signal, the effect must reach or exceed the “five-sigma” significance level, five standard deviations away from the expectation: that's a rather silly but well-established rule. But if yours gives only 3.5 or four sigma, are you allowed to get excited and wake up your boss, or should you sit back and sip your martini, with an “I know better” grin on your face? I claim the latter is a better option. You have fallen prey to the LEE: you looked in many places for a possible signal, and found a significant effect somewhere; the likelihood of finding something in the entire region you probed is greater than it would be if you had stated beforehand where the signal would be, because of the “probability boost” of looking in many places. A good rule of thumb is the following: if your signal has a width W, and if you examined a spectrum spanning a mass range from M1 to M2, then the “boost factor” due to the LEE is (M2-M1)/W. This may easily amount to a factor of 10 or 100, depending on the details of your search. An effect occurring by chance once in ten thousand cases in a given place on your spectrum may actually be just an unexciting one-in-a-hundred fluctuation! In fact, the “five-sigma” rule I mentioned above was conceived with exactly this particular effect in mind. Five sigma is a really, really rare occurrence (three in ten million), so even including the LEE, it is still something to take quite seriously. Now-a-days, we publish three-sigma results that may or may not go away with more data, and our scientific integrity requires us to account for the LEE. This is actually less easy to do than by just multiplying a probability by (M2-M1)/W as in the example above: in complex searches such as that for the Higgs boson, which combine bump hunts in many channels, this is not a trivial task. A recent paper by E. Gross and O. Vitells (Eur. Phys. J. C70:525-530,2010) has clarified some of the technical issues. The searches for the Higgs boson by CMS and ATLAS are sizing up the LEE by studying the probability of the background-only hypothesis as a function of the Higgs mass: the more the observed p-value distribution varies up and down as the signal mass hypothesis change, the stronger is the Look-Elsewhere-Effect correction that is required. Stuff for experts, for sure. But outsiders have better be aware that a three-sigma effect should not be blindly dubbed “evidence” for something new in the data. As travellers to a foreign country whose tax habits are unknown, you better ask before you buy, “LEE included or not”? — Submitted by Tommaso Dorigo
Observation of a New Particle with a Mass of 125 GeV
Observation of a New Particle with a Mass of 125 GeV lucas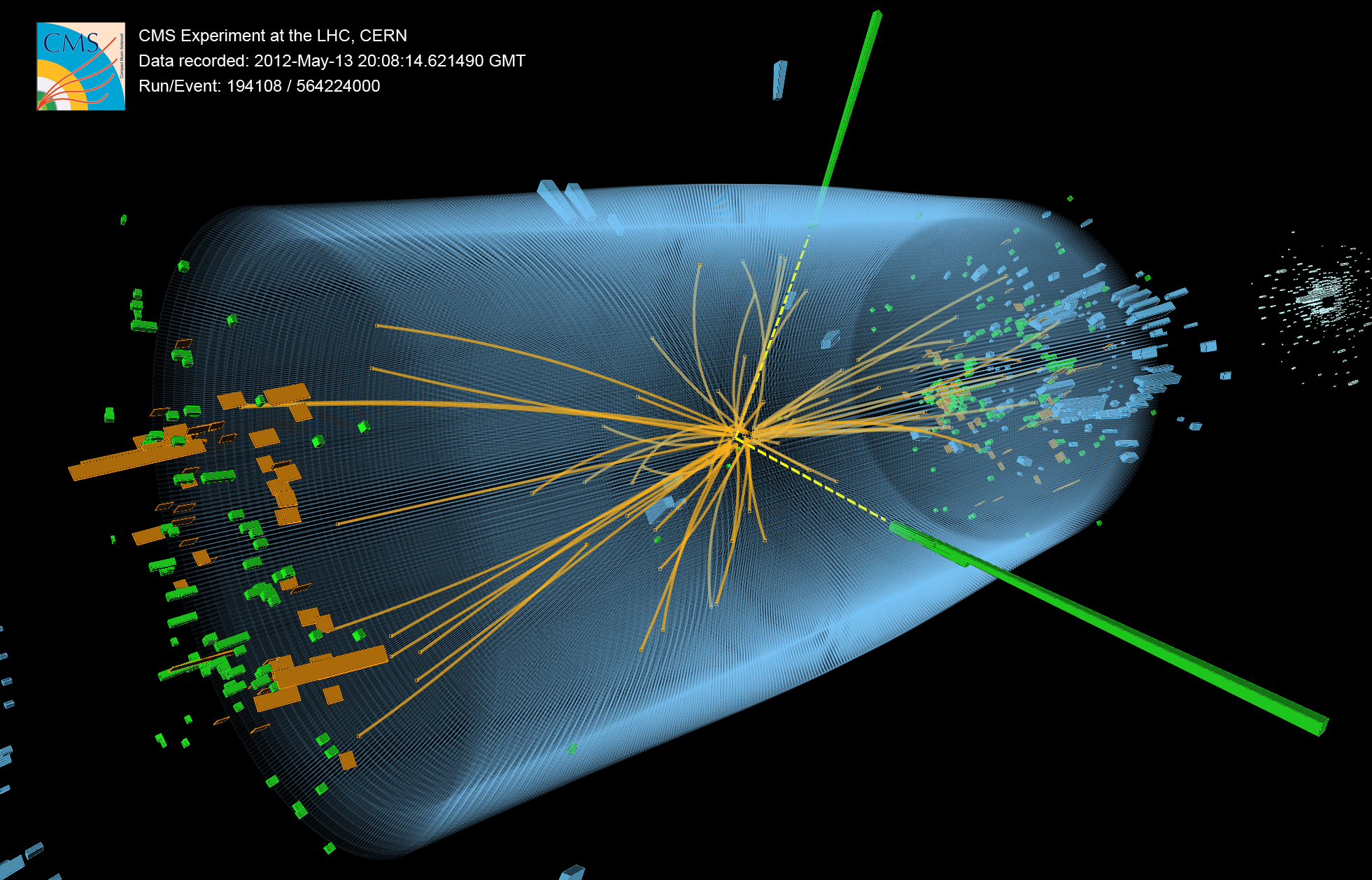
CMS Experiment, CERN
4 July 2012
The statement below is also available as PDF files in: English | French | Catalan | Chinese (traditional) | Chinese (simplified) | Croatian | Dutch | Finnish | Galician | German | Greek | Hindi | Hungarian | Italian | Korean | Persian | Polish | Portuguese | Romanian | Russian | Serbian | Spanish | Mexican Spanish | Turkish | Urdu
Summary
In a joint seminar today at CERN and the “ICHEP 2012” conference[1] in Melbourne, researchers of the Compact Muon Solenoid (CMS) experiment at the Large Hadron Collider (LHC) presented their preliminary results on the search for the standard model (SM) Higgs boson in their data recorded up to June 2012. CMS observes an excess of events at a mass of approximately 125 GeV[2] with a statistical significance of five standard deviations (5 sigma)[3] above background expectations. The probability of the background alone fluctuating up by this amount or more is about one in three million. The evidence is strongest in the two final states with the best mass resolution: first the two-photon final state and second the final state with two pairs of charged leptons (electrons or muons). We interpret this to be due to the production of a previously unobserved particle with a mass of around 125 GeV. The CMS data also rule out the existence of the SM Higgs boson in the ranges 110–122.5 GeV and 127–600 GeV with 95% confidence level[4] – lower masses were already excluded by CERN’s LEP collider at the same confidence level. Within the statistical and systematic uncertainties, results obtained in the various search channels are consistent with the expectations for the SM Higgs boson. However, more data are needed to establish whether this new particle has all the properties of the SM Higgs boson or whether some do not match, implying new physics beyond the standard model. The LHC continues to deliver new data at an impressive rate. By the end of 2012, CMS hopes to have more than triple its total current data sample. These data will enable CMS to elucidate further the nature of this newly observed particle. They will also allow CMS to extend the reach of their many other searches for new physics.
CMS Search Strategy
CMS analysed the full data sample of proton-proton collisions collected in all of 2011 and in 2012, up until June 18. These data amount to up to 5.1 fb−1 of integrated luminosity[5], at a centre-of-mass energy of 7 TeV in 2011 and up to 5.3 fb−1 at 8 TeV in 2012. The standard model predicts that the Higgs boson lasts for only a very short time before it breaks up, or “decays”, into other well-known particles. CMS studied five main Higgs boson decay channels. Three channels result in pairs of bosonic particles (γγ, ZZ or WW) and two channels result in pairs of fermionic particles (bb or ττ), where γ denotes a photon, Z and W denote the force carriers of the weak interaction, b denotes a bottom quark, and τ denotes a tau lepton. The γγ, ZZ and WW channels are equally sensitive in the search for a Higgs boson around 125 GeV and all are more sensitive than the bb and ττ channels. The γγ and ZZ channels are especially important as they both allow the mass of the new particle to be measured with precision. In the γγ channel the mass is determined from the energies and directions of two high-energy photons measured by the CMS crystal electromagnetic calorimeter (ECAL, Figure 1). In the ZZ channel the mass is determined from the decays of the two Zs to two pairs of electrons, or two pairs of muons, or a pair of electrons and a pair of muons (Figure 2). These are measured in the ECAL, inner tracking and muon detectors. The WW channel is more complex. Each W is identified through its decay to an electron and a neutrino or a muon and a neutrino. The neutrinos pass through the CMS detectors undetected, so the SM Higgs boson in the WW channel would manifest itself as a broad excess in the mass distribution, rather than a narrow peak. The bb channel has large backgrounds from standard model processes, so the analysis searches for events in which a Higgs boson is produced in association with a W or Z, which then decays to electron(s) or muon(s). The ττ channel is measured by observing τ decays to electrons, muons and hadrons.
CMS Search Results
The CMS data sample should be sensitive enough to completely exclude the mass range 110–600 GeV at 95% confidence level, if the SM Higgs does not exist. In fact, the CMS data do rule out the existence of the SM Higgs boson in two broad mass ranges of 110–122.5 GeV and 127–600 GeV with 95% confidence level. The range of 122.5–127 GeV cannot be excluded because we see an excess of events in three of the five channels analysed:
- γγ channel: the γγ mass distribution is shown in Figure 3. There is an excess of events above background with a significance of 4.1 sigma, at a mass near 125 GeV. The observation of the two-photon final state implies that the new particle is a boson, not a fermion, and that it cannot be a “spin 1” particle.
- ZZ channel: Figure 4 shows the mass distribution for the four leptons (two pairs of electrons, or two pairs of muons, or the pair of electrons and the pair of muons). Accounting also for the decay angle characteristics, it yields an excess of 3.2 sigma above background at a mass near 125 GeV.
- WW channel: a broad excess in the mass distribution of 1.5 sigma is observed.
- bb and ττ channels: no excess is observed.
The statistical significance of the signal, from a combined fit to all five channels (Figure 5), is 4.9 sigma above background. A combined fit to just the two most sensitive and high-resolution channels (γγ and ZZ) yields a statistical significance of 5.0 sigma. The probability of the background alone fluctuating up by this amount or more is about one in three million. The mass of the new particle is determined to be 125.3 ± 0.6 GeV, independent of any assumptions about the expected relative yields of the decay channels. The measured production rate (σDAT) of this new particle is consistent with the predicted rate (σSM) for the SM Higgs boson: σDAT/σSM = 0.80 ± 0.22. Great care has also been taken to understand numerous details of the detector performance, the event selection, background determinations and other possible sources of systematic and statistical uncertainties. The 2011 analysis[6] showed an excess of events at about 125 GeV. Therefore, to avoid a potential bias in the choice of selection criteria for the 2012 data that might artificially enhance this excess, the 2012 data analysis was performed “blind”[7], meaning that the region of interest was not examined until after all the analysis criteria had been fully scrutinized and approved. As a general cross-check, the analyses were performed by at least two independent teams. A number of other features reinforce confidence in the results:
- The excess is seen at around 125 GeV in both the 2011 data sample (7 TeV) and the 2012 data sample (8 TeV);
- The excess is seen at the same mass in both the high-resolution channels (γγ and ZZ);
- The excess seen in the WW is consistent with one that would arise from a particle at 125 GeV;
- The excess is seen in a range of final states involving photons, electrons, muons and hadrons.
The preliminary results presented today will be refined, with the aim of submitting them for publication towards the end of the summer.
Future Plans
The new particle observed at about 125 GeV is compatible, within the limited statistical accuracy, with being the SM Higgs boson. However, more data are required to measure its properties such as decay rates in the various channels (γγ, ZZ, WW, bb and ττ) and ultimately its spin and parity, and hence ascertain whether it is indeed the SM Higgs boson or the result of new physics beyond the standard model. The LHC continues to perform extremely well. By the end of 2012, CMS expects to more than triple its total data sample, and hence to probe further the nature of this new particle. If this particle is indeed the SM Higgs boson, its properties and implications for the standard model will be studied in detail. If it is not the SM Higgs boson, CMS will explore the nature of the new physics that it implies, which may include additional particles that are observable at the LHC. In either case, searches will also continue for other new particles or forces that can be observed in future runs of the LHC at higher beam energies and intensities.
More information
- This statement is available online at: http://cmsexperiment.web.cern.ch/news/observation-new-particle-mass-125-gev
- CMS public website: http://cern.ch/cms
- CMS Higgs Seminar at CERN (Prof. Joseph Incandela)
- CERN Press Release, 4 July 2012 [ Also available in French ]
- ATLAS Experiment Higgs search results
- ATLAS Higgs Seminar at CERN (Prof. Fabiola Gianotti)
- Full video of the seminar
- Full video of the press conference
- Photographs from the seminar and press conference
- Photographs from the webcast at ICHEP
Event images and animation of real CMS collisions
Images:
- Two photons i.e. γγ (8 TeV) event display
- ZZ to two electrons and two muons (8 TeV) event display
- All images from this statement (including plots)
Animations:
- Two photons i.e. γγ (8 TeV) event display animation: CDS | YouTube
- ZZ to two electrons and two muons (8 TeV) event display animation: CDS | YouTube
- ZZ to 4 muons (7 TeV) event display animation: CDS | YouTube
Short movies about the Higgs
- Animation of the Higgs mechanism [with subtitles] See also [without subtitles]
- What is the Higgs? by Don Lincoln
- Higgs boson: How do we search for it? by Don Lincoln
References
More about the Higgs at CMS
- CMS Physics Analysis summary papers about the Higgs
- About the Higgs boson
- Simple explanations of Higgs search terminology
- Search for the Standard Model Higgs boson in CMS
- All CMS Higgs plots and results
About CMS Physics
- All CMS Papers
- All CMS Physics Analysis Summaries
- All CMS results
- Images of real collisions in CMS
- Animations of real collisions in CMS
About CMS
More information: http://cern.ch/cms, or contact: cms.outreach@cern.ch. CMS is one of two general-purpose experiments at the LHC that have been built to search for new physics. It is designed to detect a wide range of particles and phenomena produced in the LHC's high-energy proton-proton and heavy-ion collisions and will help to answer questions such as: "What is the Universe really made of and what forces act within it?" and "What gives everything mass?" It will also measure the properties of well-known particles with unprecedented precision and be on the lookout for completely new, unpredicted phenomena. Such research not only increases our understanding of the way the Universe works, but may eventually spark new technologies that change the world in which we live as has often been true in the past. The conceptual design of the CMS experiment dates back to 1992. The construction of the gigantic detector (15 m diameter by nearly 29 m long with a weight of 14000 tonnes) took 16 years of effort from one of the largest international scientific collaborations ever assembled: 3275 physicists (including 1535 students) plus 790 engineers and technicians, from 179 institutions and research laboratories distributed in 41 countries all over the world.
Footnotes
[1] ICHEP is the 36th International Conference on High Energy Physics, Melbourne, Australia during 4-11 July, 2012. The results will be presented jointly: in person at CERN and by real-time video link to ICHEP. [2] The electron volt (eV) is a unit of energy. A GeV is 1,000,000,000 eV. In particle physics, where mass and energy are often interchanged, it is common to use eV/c2 as a unit of mass (from E = mc2, where c is the speed of light in vacuum). Even more common is to use a system of natural units with c set to 1 (hence, E = m), and use eV and GeV as units of mass. [3] The standard deviation describes the spread of a set of measurements around the mean value. It can be used to quantify the level of disagreement of a set of data from a given hypothesis. Physicists express standard deviations in units called “sigma”. The higher the number of sigma, the more incompatible the data are with the hypothesis. Typically, the more unexpected a discovery is, the greater the number of sigma physicists will require to be convinced. [4] Confidence level is a statistical measure of the percentage of test results that can be expected to be within a specified range. For example, a confidence level of 95% means that the result of an action will probably meet expectations 95% of the time. [5] http://news.stanford.edu/news/2004/july21/femtobarn-721.html [6] http://cmsexperiment.web.cern.ch/news/cms-search-standard-model-higgs-boson-lhc-data-2010-and-2011 [7] http://cmsexperiment.web.cern.ch/news/blinding-and-unblinding-analyses