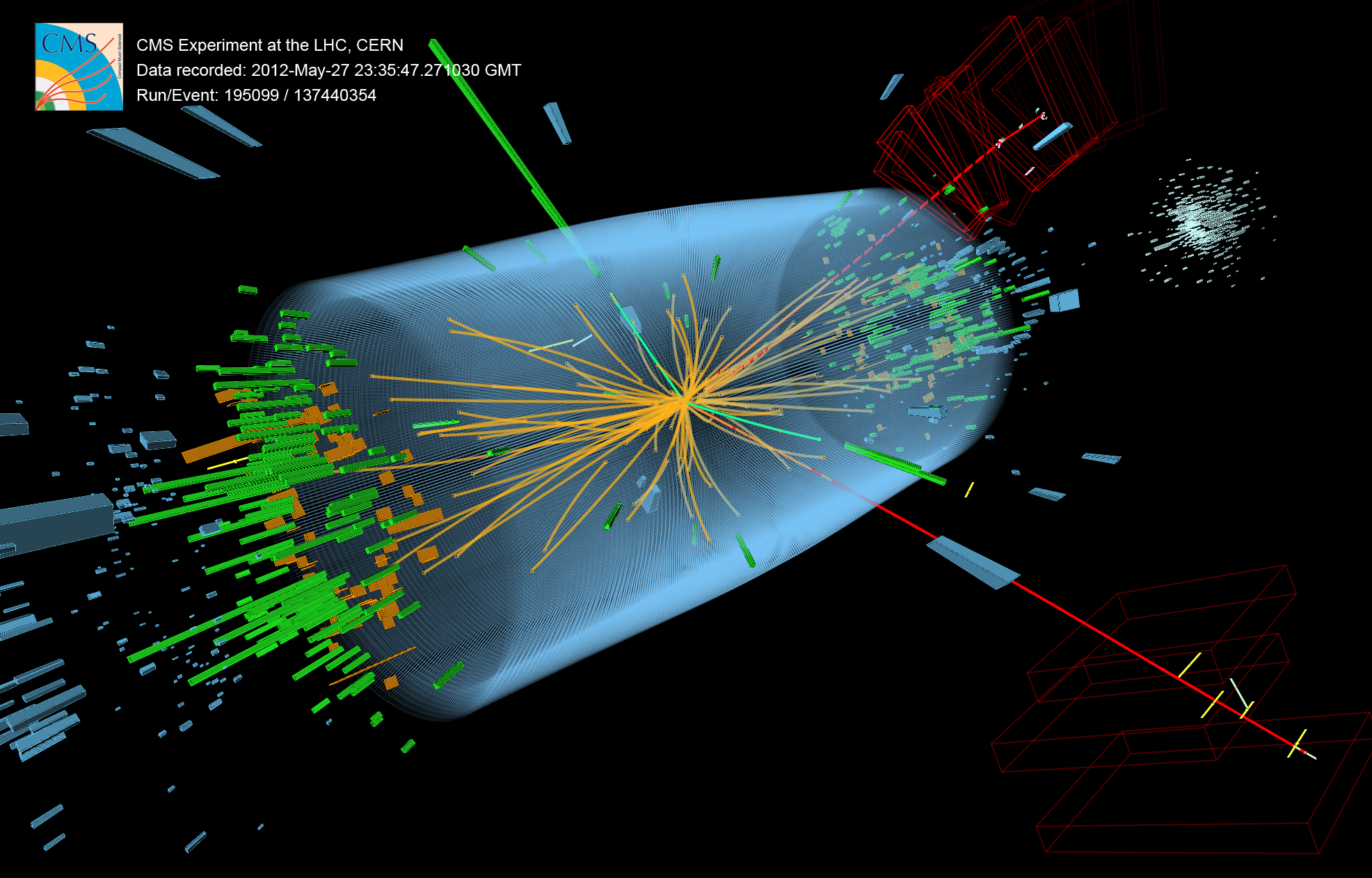
CMS is presenting a wide range of results for the annual winter conference, Rencontres de Moriond. The first week of Moriond — 3–8 March — is devoted primarily to electroweak (EW) studies. Among the CMS results are further studies of the recently discovered Higgs boson candidate, searches for new physics beyond the Standard Model and precision measurements of the properties of the top quark. We present below brief highlights from a few of the many excellent analyses that will be shown at the conference.
Higgs decays into pairs of Z bosons, W bosons or τ (tau) leptons
In July 2012, CMS and ATLAS announced the discovery of a new particle consistent with the predictions of the Standard Model (SM) Higgs boson. However, in order to confirm whether the particle is indeed the expected Higgs boson requires a more thorough understanding of its properties. The following results all take into account around 5 fb−1 and 20 fb−1 (inverse femtobarn[1]) of proton-collision data recorded by CMS at centre-of-mass energies of 7 and 8 TeV[2], respectively.
Decays to two Z bosons:
HIG-13-002 The study of the new boson’s decay into two Z particles is crucial to the determination of two of its quantum numbers, spin and parity; the Standard Model predicts that the Higgs boson should have zero spin and positive parity (represented as 0+). CMS recently published a study showing that the data were consistent with 0+ and excluded 0− at 97% confidence level (Phys. Rev. Lett. 110, 081803 (2013)). The data collected by CMS have now been used to show that the boson’s spin-parity is even less consistent with 0− (excluded with greater than 99.8% confidence) and is also not consistent with 2+ (excluded with greater than 98.5% or 99.9% confidence for the simplest 2+ model with production modes via gluons or quarks respectively), 1+ or 1− (each of which is excluded with greater than 99.9% confidence for exotic spin-1 models)*. Also, in July 2012, combining the ZZ and γγ (two-photon) channels gave CMS an observation with a significance of 5σ[3]. Now, using the full dataset collected until the end of 2012, the ZZ channel alone gives the observation of the boson a significance of 6.7σ. The mass of the new particle has also been measured precisely in this channel, giving us a value of 125.8 GeV. The new results include the measurement of the production mechanism of this particle, and the rate of production with subsequent decay into two Z bosons divided by the expected rate for a SM Higgs gives a ratio (μ) of around 0.91, suggesting that the particle behaves like a SM Higgs. In addition, CMS has excluded the existence of the SM Higgs boson in the range 130–827 GeV at 95% confidence level[4].
Decays to two W bosons:
HIG-13-003 This channel studies the decays of the Higgs boson candidate into two W bosons, each of which subsequently decay into a lepton and a neutrino. As the neutrino cannot be detected directly by CMS, the resolution of this channel is not as sharp as that of the ZZ or γγ channels. From studies of the full collected dataset, CMS excludes the SM Higgs boson in the mass range of 128–600 GeV at 95% confidence level, while observing an excess at around 125 GeV with a significance of 4σ.
Decays to two τ leptons:
HIG-13-004 The electroweak-symmetry breaking mechanism (popularly known as the Brout-Englert-Higgs mechanism) was proposed to explain how the heavy bosons (Ws and Zs) got their masses. However, this mechanism can also explain how elementary fermions (quarks or leptons) acquire mass. An important test of whether the discovered boson is indeed the SM Higgs is to observe its decay into fermions. CMS performed a search for the decay of the new boson into τ leptons. When the discovery announcement was made in July 2012, the observation in this channel was compatible with both the existence and the absence of a SM Higgs. Since then, with the additional data recorded by CMS, a clear signal in this channel has emerged for a boson with a mass of around 125 GeV, corresponding to a significance of 2.8σ, lending further credence to the expectation that the particle discovered behaves like a SM Higgs boson.
Searches for new physics
The Standard Model of particle physics has stood tall for decades, with successful prediction after successful prediction. However, we know that this is not the whole story and that new physics — that which is beyond the Standard Model — must exist out there. The LHC is a discovery machine, and should such new physics exist within the energies that can be probed here, we expect to find it.
The search for SUSY:
SUS-12-024, SUS-13-007, SUS-13-003 One of the most promising extensions of the Standard Model is known as Supersymmetry (or SUSY for short). SUSY predicts that for every boson there exists a fermionic superpartner and vice versa. CMS has performed many direct and indirect searches for these “sparticles”. Previous searches, utilising 5 fb−1 of data collected at a centre-of-mass energy of 7 TeV in 2011, yielded no top or bottom squarks (the superpartners of the top and bottom quark, respectively, also known as stops and sbottoms). CMS has now studied the entire dataset from 2012 (~20 fb−1, 8 TeV) looking for these sparticles. If they are produced, we expect stops and sbottoms to decay into bottom quarks, producing particle jets (b-jets, in this case). These decays are also expected to produce the lightest SUSY particle (LSP), which might leave no trace in the detector and will be characterised only by large missing energy. CMS therefore looked for an excess in events with multiple particle jets — at least one of which is a b-jet — as well as 1) large missing energy, or 2) at least one lepton, or 3) multiple leptons. The observations are consistent with the Standard Model and show no new physics in this domain with 95% confidence levels. In events with large missing energy, CMS has ruled out gluinos with a mass of up to 1.15 TeV, for LSP masses of less than 500 GeV; in events with leptons, CMS has excluded the existence of gluinos up to 1.3 TeV, for LSP masses of less than 500 GeV.
Searches for new, “exotic” physics:
EXO-12-026 Some physics theories predict the existence of exotic charged particles that live long enough to traverse the CMS detector, if they were to be produced in a proton collision. These quasi-stable particles — known as heavy, stable, charged particles or HSCPs — may have unit charge (like an electron or proton; |Q|=1e), fractional charge (|Q|<1e), or be multiply charged (|Q|>1e). Some sparticles are also expected to belong to the HSCP family, so this search also includes searches for long-lived SUSY particles. Most particles produced due to Standard Model processes will fly through CMS at nearly the speed of light (~c). However, HSCPs often travel considerably slower (<0.9c). Intriguingly, HSCPs may start out charged and then turn neutral (so they leave tracks only in the inner Tracker of CMS and not the outer muon system), may start out neutral and then acquire charge (so they leave no tracks in the Tracker but only in the muon system), or may retain their charge throughout their journey inside the CMS detector (with tracks in both the Tracker and the muon system). In the absence of significant excesses in these searches, CMS has placed the most stringent limits on the existence of a variety of predicted particles ranging from gluinos and stops to fractionally and multiply charged particles.
Searches for new resonances:
EXO-12-060, EXO-12-061, EXO-12-059 The Higgs-like boson was discovered by CMS and ATLAS by looking for resonances — an excess of particles produced at a certain mass in a range of masses. This method is also used to search for other new particles, such as heavier W and Z bosons, known as W′ (W-prime) and Z′ (Z-prime) respectively. These searches were performed in a variety of channels: single lepton plus missing energy (W′), di-lepton (Z′), and di-jets (W′, Z′ and a host of other particles such as excited quarks and gravitons), utilising around 20 fb−1 of proton collision data collected at a centre-of-mass energy of 8 TeV in 2012. CMS observed no excess in the lepton-and-missing-energy channel between 300 GeV up to 3.35 TeV, excluding the existence of one particular type of W′ in this mass range with 95% confidence level. Similarly, no excess was observed in the di-lepton channel, ruling out two different kinds of Z′ with 95% confidence levels up to 2960 GeV and 2600 GeV respectively, the most stringent limits placed on their masses so far. The search in the di-jet channel extends, depending on the particles in question, all the way from 1.20 TeV up to 1.58–5.08 TeV; note that CMS had previously excluded the existence of these particles for masses below 1.2 TeV.
Top-quark physics
The top quark was discovered at Fermilab’s Tevatron in 1995, some 22 years after its existence was predicted. Its discovery completed the three quark families of the Standard Model, and today it plays an important role in many physics searches at the LHC. Since tops are produced abundantly at the LHC, they form a crucial background in many measurements, and a thorough understanding of the top quark’s properties is paramount in describing this background.
Ratio of branching fractions:
TOP-12-035 The top quark is the heaviest of all observed quarks, and decays almost instantly into a down-type quark (a down quark, a bottom quark or a strange quark) and a W boson. Theory predicts that it would decay into a bottom quark 99.9% of the time (known as the t→bW branching fraction). Therefore, the ratio (R) of the branching fraction to bottom quarks (t→bW) and the branching fraction to all three types of quarks (t→qW; where q = d, b or s) should be close to 0.999. For this measurement, CMS used around 17 fb−1 of 8 TeV data collected in 2012. By counting the number of jets produced in the detector associated with a bottom quark (b-jets), CMS has calculated this ratio to be 1.023+0.036−0.034, the most precise measurement of this quantity to date.
Dependence of top-quark mass on other observables:
TOP-12-029 Quarks carry the so-called colour charge, which means they are attracted by the strong force and cannot be observed as free particles. When a top quark decays into a bottom quark, strong colour-force-fields are created, building up enough energy to create new quark pairs and other particles in the process. This “slows down” the top quark and the particles it created, changing the final pattern of particles observed in the CMS detector. To measure the mass of the top quark, you have to carefully measure all of the particles produced in its decay. However, this measurement may get altered to some extent by the force of the colour fields. The exact size of this effect though cannot be calculated very precisely and is therefore unknown. To probe experimentally the possible effects of such colour fields on the top quark’s mass measurement, CMS has determined for the first time how the measured top mass depends on observables in a collision event that are related to the configuration of the colour fields. Analysing 5 fb−1 of data recorded in 2011, it was found that variations in the measured top mass as a function of the event observables are well modelled by the existing event simulations, excluding possible dramatic effects. This measurement is a first step towards an improved understanding of the colour fields in top-quark events, which will ultimately allow CMS to measure the top-quark mass with improved precision, and to better model top-quark events as a background to searches for new physics at the LHC. * Typographical errors in the values shown here were corrected on 6 March at 15:30 CET.
More information
- This statement is available online at: http://cmsexperiment.web.cern.ch/news/new-cms-results-moriond-electroweak-2013
- CMS public website: http://cern.ch/cms
- CERN Press Release, 1 March 2012
Animation of real CMS collisions
Animations:
- (Higgs) ZZ to two electrons and two muons (8 TeV) event display animation: CDS | YouTube
- (Higgs) ZZ to 4 muons (7 TeV) event display animation: CDS | YouTube
Short movies
- Animation of the Higgs mechanism [with subtitles] See also [without subtitles]
- What is the Higgs? by Don Lincoln
- Higgs boson: How do we search for it? by Don Lincoln
- John Ellis on SUSY
References
- Simple explanations of Higgs search terminology
- Explanation of the Higgs boson exclusion plots (on atlas.ch)
- Blinding and unblinding analyses
CMS Physics
- All CMS Papers
- All CMS Physics Analysis Summaries
- All CMS results
- Images of real collisions in CMS
- Animations of real collisions in CMS
- Receive updates on latest CMS papers and more | Follow @CMSpapers on Twitter
About CMS
More information: http://cern.ch/cms, or contact: cms.outreach@cern.ch. CMS is one of two general-purpose experiments at the LHC that have been built to search for new physics. It is designed to detect a wide range of particles and phenomena produced in the LHC's high-energy proton-proton and heavy-ion collisions and will help to answer questions such as: "What is the Universe really made of and what forces act within it?" and "What gives everything mass?" It will also measure the properties of well-known particles with unprecedented precision and be on the lookout for completely new, unpredicted phenomena. Such research not only increases our understanding of the way the Universe works, but may eventually spark new technologies that change the world in which we live as has often been true in the past. The conceptual design of the CMS experiment dates back to 1992. The construction of the gigantic detector (15 m diameter by nearly 29 m long with a weight of 14000 tonnes) took 16 years of effort from one of the largest international scientific collaborations ever assembled: 3275 physicists (including 1535 students) plus 790 engineers and technicians, from 179 institutions and research laboratories distributed in 41 countries all over the world.
Footnotes
[1] http://news.stanford.edu/news/2004/july21/femtobarn-721.html [2] The electron volt (eV) is a unit of energy. A GeV is 1,000,000,000 eV; a TeV is 1000 GeV. In particle physics, where mass and energy are often interchanged, it is common to use eV/c2 as a unit of mass (from E = mc2, where c is the speed of light in vacuum). Even more common is to use a system of natural units with c set to 1 (hence, E = m), and use eV and GeV as units of mass. [3] The standard deviation describes the spread of a set of measurements around the mean value. It can be used to quantify the level of disagreement of a set of data from a given hypothesis. Physicists express standard deviations in units called “sigma”. The higher the number of sigma, the more incompatible the data are with the hypothesis. Typically, the more unexpected a discovery is, the greater the number of sigma physicists will require to be convinced. [4] Confidence level is a statistical measure of the percentage of test results that can be expected to be within a specified range. For example, a confidence level of 95% means that the result of an action will probably meet expectations 95% of the time.
- Log in to post comments